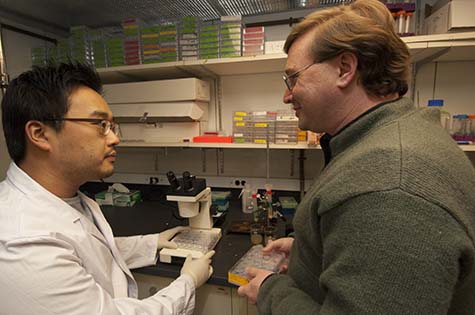
If you pull up a soybean or bean plant and shake off the dirt, you might see odd swellings or bumps, like rheumatic finger joints, on its roots. Inside the cool, soil-covered bumps are bacteria that are making nitrogen with the help of an enzyme, something chemical factories can do only with the help of a catalyst and at high temperature and pressure.
The bacteria, typically members of the genus Rhizobia, break the strong triple bond between the nitrogen molecules in the air and repackage the nitrogen atoms in chemical compounds the plant can use. In return, the plant supplies the bacteria with the energy needed to split the nitrogen molecules in the form of sugar.
Legume-Rhizobia partnerships generate more nitrogen for plants than all industrial fertilizers used today, and they provide the right amount of nitrogen at the right time.
By contrast, much of the synthetic fertilizer applied to farm fields is wasted, washing out the soil into waterways or evaporating into the atmosphere in the form of nitrous oxide, where it becomes an environmental and health risk.
Farmers can already buy Rhizobia-rich biofertilizers to increase nodule formation and improve soil quality without synthetic fertilizers. But scientists are beginning to talk about re-engineering crop plants so that, like legumes, they will have on-site nitrogen-fixing systems, either in root nodules or in the plant cells themselves.
To do that, scientists need to understand the biological nitrogen-fixing machinery as thoroughly as a mechanic understands the valves and pistons of a car engine. The difference is that the biological machinery is far too small to be visible to the unaided eye.
Science got one step closer to this goal recently when a team at Washington University in St. Louis worked out the structure of a protein called NolR that acts as a master off-switch for the nodulation process. By building an accurate atomic model of the protein, they were able to “see” exactly how it recognizes and slots itself into genes to prevent bacteria from embarking on a life as a symbiont. The work was published in April 29 issue of PNAS,
Trust but verify
The process of nodulation is so weird that if you read about it in a science fiction book, you’d probably credit the author with a great imagination.
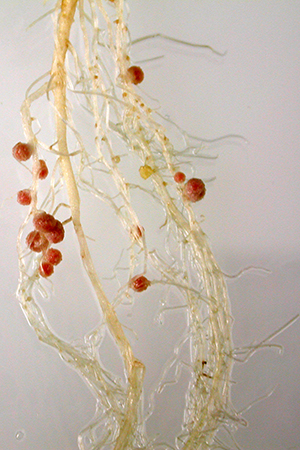
First, the plant and soil bacteria engage in a molecular dialog to make sure they are compatible partners. The host plant releases a cocktail of chemicals called flavonoids that are perceived by a bacterial protein named NodD, which turns on nod (nodulation) genes. Together the nod genes make a large, complicated molecule called a nod factor.
The nod factor triggers the plant to make an“infection thread,” or a tube through which the bacteria travel deep into the root, where they are wrapped in membrane the plant has synthesized and sequestered in vesicles within the root cortex cells of a nodule. Their metabolism and even their ability to reproduce are so altered that they are like different organisms, and are called bacteroids rather that bacteria.
“It’s like a pathogen invasion,” said Joseph Jez, PhD, associate professor of biology in Arts & Sciences at Washington University, “but Rhizobium is not a pathogen, it’s a beneficial organism. “
Does it curl or lie flat?
Scientists working on the genetics of nodule formation in the mid-1980s and early ’90s, identified NodD, a master on-switch for nod genes, and NolR, a master off- switch that acts even more broadly, turning off the nod genes, NodD, and other genes needed for life as a symbiont.
“The bacteria has the ability to turn on a bunch of genes for nodulation and the symbiosis, but it needs to keep them turned off as long as it is free-living,” Jez said. “And that’s the role of NolR.”
“It’s like driving a car,” said Soon Goon Lee, PhD, postdoctoral research associate in the Jez lab. “NodD is the gas pedal and NolR is the brake. But we didn’t know how NolR worked until we solved its structure and could understand how it interacted with DNA.”
The job is to figure out how the long, thread-like protein molecule folds on itself to form a snarl of helices and ribbons, and then how the folded molecule fits into and binds with the DNA.
Unfortunately, protein folding is a notoriously hard problem, one as yet beyond the reach of computer calculations. So most protein structures are still solved by the time-intensive process of crystallizing the protein and then barraging the crystal with X-rays to locate the atoms within it.
In 2005, Hari R. Krishnan, PhD, a U.S. Department of Agriculture scientist at the University of Missouri-Columbia, told Jez that he had a NolR clone, or population of cells that all made the NolR protein. Would Jez be interested in trying to crystallize NolR so that its structure could be solved, Krishnan asked.
Jez happily accepted, and, as time permitted, he or another member of the lab would put NolR through a crystallization screen, depositing drops of the protein into little wells filled with different solutions, searching for conditions that would cause the protein to crystallize.
At first, NolR was uncooperative. If they got crystals, the crystals fell apart when they were manipulated or they produced diffraction patterns that couldn’t be interpreted.
In his turn, Lee picked up the project. He decided to start at the beginning again, picking a DNA sequence NolR bound, ordering that snip of DNA, and then trying to crystal a mixture of the protein and DNA together.
This should have been harder than crystallizing the protein alone, but to his surprise it turned out to be easier. The low-resolution data Lee got for the protein/DNA complex made it easier for the scientists to interpret the high-resolution data from the protein alone.
The protein turned out to have what is called a helix-turn-helix motif commonly found in proteins that bind DNA. The DNA double helix has a major groove and minor groove that run down the double helix like the threads of a screw. Many proteins that bind to DNA do so through the wider major groove.
The helix-turn-helix motif of the protein NolR consists of alpha helices (pale blue and pink) separated by a short string of amino acids (the turn). The helices are spaced to fit into the major groove of a DNA molecule (the white and gray double helix below NolR) and bind to “consensus sequences” in the DNA (purple and red), common genetic sequences found in all the genes NolR binds.
“The major groove is the one that’s open, and you can fit a protein helix into that groove,” Jez said. “So nature uses this helix-turn-helix domain as a way of positioning helices into the major grooves. The protein is a dimer, so it has two helices that are spaced perfectly to put one into each of two consecutive major grooves.”
To act as a master switch, NolR has to be able to recognize and bind to many different genes. It’s able to do that because each of the genes carries the same sequence of nucleotides, called a consensus sequence, somewhere along its length. In this case, there are two such sequences in consecutive major grooves in all the genes NolR binds.
The scientists are pleased with their progress but it has only made them more eager to crystallize the other protein: the on-switch, NodD. After all, it’s hard to drive a car when you only have a brake but no accelerator.